Understanding current loop output sensors
For analog sensor data transmission, a 4-20 mA current loop is a very common method to convey the sensor data acquired. Sensors or transducers are usually designed to measure a range of values of the measured parameter, which is known as the measurand. The measurand value must be converted to current within the measuring device in such a way that the current in the loop will be proportional to the measurand value. The range of the loop current, 4 mA to 20 mA, is called the span of the transmitter. The transmitter is typically configured so that one end point of the measurement value will correspond to 4 mA and the other end point value measured will correspond to 20 mA.
The 4-20 mA current loop has become the standard for signal transmission and electronic control in most analog control systems. A 4-20mA current loop circuit is shown in Figure 1. In a current loop, the current is drawn from a DC loop power supply, then flows through the transmitter using field wiring connected to a loop load resistor in the receiver or controller, and then back to the loop supply, with all elements being connected in a series circuit. All current-loop-based measuring systems use at least these four elements.
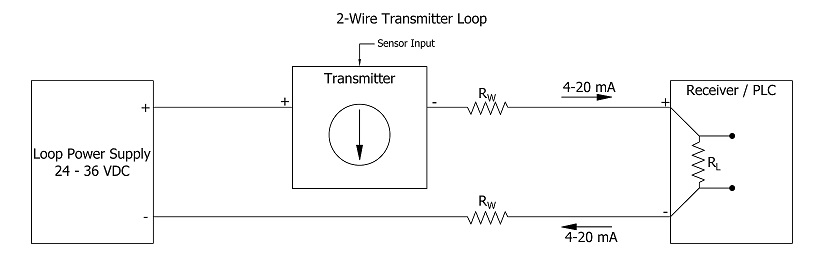
Figure 1.Typical 4-20 mA current loop
Advantages of a Current Loop
An obvious question arises: Why use a 4-20 mA current loop to transmit the analog data from a sensor? The answer is that a 4-20 mA current loop offers several benefits for such sensor data transmission:
- A major reason is that the loop current does not vary with long field wiring, as long as the voltage developed in the loop, called the Compliance Voltage, can sustain the maximum loop current.
- Another benefit is that the current loop has a low impedance and is not particularly susceptible to noise or EMI at large.
- A third advantage is the live-zero feature of the loop (the 4 mA low limit), which makes the loop self-diagnostic if there is a break or bad connection in the loop or a loop power supply failure.
- A current loop permits other current operated devices such as a remote readout or a recorder to be put in series with the loop, within the constraints permitted by the loop's Compliance Voltage.
- The low level of maximum loop current (20 mA) allows the use of relatively simple safety barriers to limit loop current to an Intrinsically Safe level that prevents ignition in a Hazardous Location.
Loop Power Supply and Compliance Voltage
When current is transmitted in the loop, there are voltage drops due to the field wiring conductors and any connected devices. However, these voltage drops do not affect the current in the loop as long as the total loop voltage is sufficient to maintain the maximum loop current. The element responsible for maintaining a stable current in the loop (as shown in Figure 1) is the loop DC power supply. The range of voltage over which the loop will function is called its Compliance Voltage. Common values for 4-20 mA loop supplies are 24VDC or 36VDC. The voltage chosen by a designer depends on the number of elements connected in series with the loop, because the loop power supply voltage must always be higher than the sum of all the voltage drops in the circuit, including the field wiring voltage drop. The sum of all these voltage drops is known as the loop's minimum compliance voltage. There are certain requirements that the compliance voltage must be able to fulfill, the two most important of which are:
- The loop power supply voltage must be able to power all the devices in the loop, including the field wiring's voltage drop, when the current is at its maximum value, normally 20 mA.
- The loop power supply maximum voltage output must be equal to or lower than the maximum voltage rating of any device in the loop.
Transmitter
A sensor or transducer that measures a physical parameter, such as temperature, pressure, position, or fluid flow is connected to a signal conditioning circuit that converts the measured parameter value to an electrical output signal such as a voltage or current proportional to the measured physical parameter. If this electrical signal is a 4-20 mA DC output connected into a current loop, the hardware and electronics system which sends this current into the loop is called a transmitter. A transmitter may consist of a single device containing a sensing element and internal electronics, or it may utilize a sensor or transducer connected to separate signal conditioning electronics configured as a 4-20 mA current transmitter.
Field Wiring
The 4-20 mA current circulates in the loop. The distance between the sensor-transmitter combination and the process controller or readout can be several hundreds of feet or more. Field wiring conductors are used in the loop to connect the transmitter to the process monitoring or control hardware. It is important to see them as an element of the loop because they have some resistance and produce a voltage drop, just like any other element in the loop. If the sum of all the voltage drops is higher than the loop power supply compliance voltage, the current will not be proportional to the measured parameter and the system will produce unusable data.
The resistance of the field wiring conductors is normally given in Ohms per length, typically Ohms per 1000 feet, so the total resistance is the product of this value times the length of the wires divided by 1000. Note that the wire length includes the loop conductor going to the receiver and the loop conductor for the current return from the receiver, the sum of which is twice the individual conductor length. The total field wiring resistance is represented by the symbol Rw, as is shown in the diagram. The voltage drop due to the field wiring is given by Ohm’s law as:
Where I is in Amperes; Rw is in Ohms; and Vw is in Volts.
Receiver or Process Controller
After the loop current is generated, it must usually be further processed in the system.. For example, the current could be used as feedback to a valve controller to open, close, or modulate the valve in order to initiate or control a process. It is generally easier to perform control functions with a voltage rather than a current. The receiver is the the part of the loop circuit that converts the loop current into a voltage. In Figure 3, the receiver is a simple resistor that is in series with the loop, so from Ohm's Law, the voltage developed across it is directly proportional to the loop current, which is itself proportional to the measured physical parameter, the measurand.
Loop Load Resistor
The load resistor used in a 4-20 mA current loop is not an arbitrary value. For any specified compliance voltage, there is a maximum loop load resistance that will permit full current to be developed in the loop. Exceeding the maximum loop resistance, which must include the resistance of the field wiring, prevents the system from providing the full 20 mA output current in the loop. In the case of a typical current output sensor, whose loop load graph is shown in Figure 2 below, at 18 Volts input, the total loop load can be as high as 550 Ohms. At 24 Volts input, total loop load can be as high as 850 Ohms, and at the system's maximum input of 32 volts, the total loop load can be 1200 Ohms.
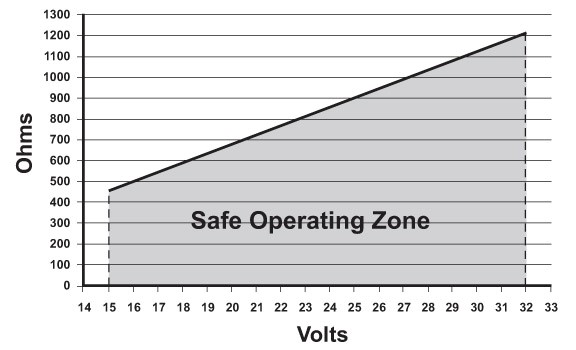
Figure 2 Loop load resistance vs. loop supply voltage for a typical current loop output sensor.
The Importance of Choosing the Right Loop Load Resistor
The choice of the loop load resistor usually depends on the input signal voltage the receiver system requires for good resolution. A 4-20 mA loop current will develop 2-10 VDC across a 500 Ohm load resistor (E = IR). If the receiver system will work satisfactorily with a lower input voltage, the 4-20 mA loop current will develop 1-5 VDC across a 250 Ohm load resistor, which is the most common loop load. Note that a loop load resistor is quite often already built into the receiver input terminal connections. Check the specifications of the receiving device to determine if there is a loop load resistor supplied at its input..
It is very important that the loop load resistor wattage rating is sufficient to ensure that any heating caused by current flowing through the resistor won't change the resistor's value and thereby change the voltage developed across it. Recall that the wattage dissipated by the resistor is I² x R. For a 500 Ohm load resistor, the power dissipated at 20 mA is 0.2 Watts. A good choice for the resistor's power rating is at least 2 Watts because such a load will not heat up very much. Even at full loop current there won’t be a voltage change across the load resistor due to heat from power dissipation instead of actual loop current changes. Note that wire-wound resistors usually have lower temperature coefficients than metallized resistors.
Types of Transmitters
There are several different varieties of current transmitters used for 4-20 mA current loops. In general, they conform to the following categories, delineated by the number of connections required for operation:
- 2-wire transmitters, which usually function as loop-powered current sinking devices.
- 3-wire transmitters, which are independently-powered loop current sourcing devices.
- 4-wire transmitters, which are normally independently-powered devices used when loop isolation is needed for noise or ground loop elimination, or for operation in hazardous locations (hazlocs).
- Derivatives of 4-wire transmitters such as loop isolators or current loop repeaters. Often such devices are incorporated into a national-agency-approved safety barrier for intrinsically safe (IS) systems that can be safely operated in a code-specific hazardous location environment.
2-Wire Current Loop Powered Transmitters
2-wire loop-powered transmitters are electronic devices that can be connected in a current loop without having a separate or independent power source. They are designed to take their power from the current flowing in the loop. Typical loop-powered devices include sensors, transducers, transmitters, repeaters, isolators, meters, recorders, indicators, data loggers, monitors, and many other types of field instruments.
Loop-powered devices are important because for some systems it is difficult to supply separate power to all the devices and instruments in the loop. The device might be located in an enclosure where access might be difficult, or in a hazardous location (hazloc) where power cannot be allowed or must be limited.
Figure 3 shows a 2-wire loop-powered device connected to a current loop. It is considered a current sinking device in the loop circuit. The power to operate the device is supplied entirely by the unused current below 4 mA in the loop. 2-wire loop powered transmitters are popular, but are usually more costly than 3-wire transmitters.
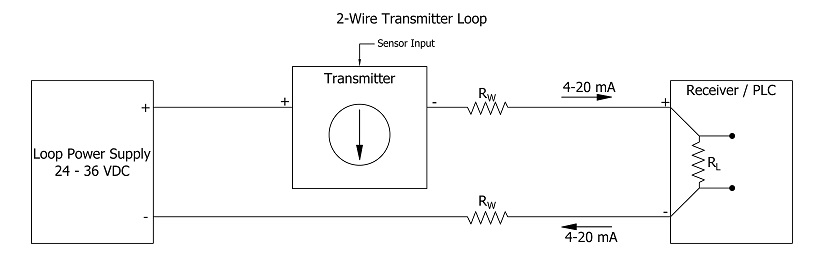
Figure 3 Typical 2-wire loop powered system
3-Wire Current Transmitters
3-wire transmitters are different from loop-powered transmitters because their loop current is developed from a DC power supply that supplies more current than just the loop current. The entire transmitter operates off this supply, and may consume much more current than typical 2-wire loop powered devices. However, a 3-wire system is a sourcing element, so it supplies the current loop despite what it uses itself. 3-wire transmitters are often less costly than 2-wire. A typical 3-wire loop is shown in Figure 4 below. It is important to note that a 3-wire transmitter cannot be connected into a 2-wire loop powered system without significant loop circuit reconfiguration.
Notice the high side of the power supply is not directly connected to the loop, but that the return side of the power supply is connected via a grounded point, so a 3-wire transmitter requires careful consideration of grounding issues to prevent potential ground loops. If an application using a 3-wire transmitter requires isolation in the loop, there are several paths to follow.
One way is to use a separate DC power supply for each 3-wire loop output device, so that there is no interaction with other current loops. Another way is to use a loop isolator module. These devices utilize various methods to achieve galvanic isolation, typically using transformers or optical couplers. They accept a 4-20 mA signal, function as a repeater or re-transmitter, and deliver a reconstituted 4-20 mA current loop signal that is fully isolated. A third way is to use a 4-wire transmitter which has isolation already built in.
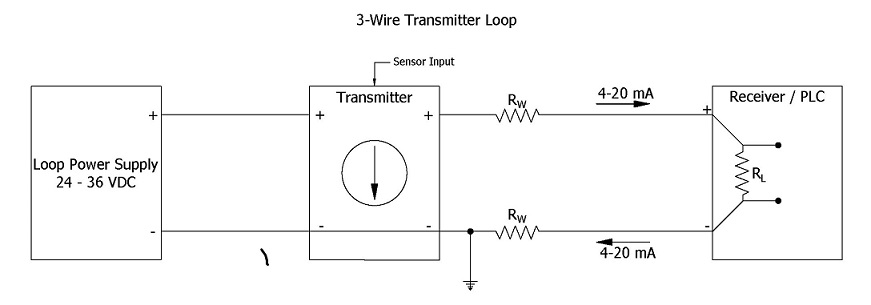
Figure 4 Typical current loop using a 3-wire transmitter
4-Wire Current Transmitters
4-wire transmitters offer the current sourcing advantages of a 3-wire device, but also provide galvanic isolation for the current loop output. 4-wire devices are substantially more expensive than 3-wire devices. For this reason, they are generally used where the isolation is needed, or they are part of a combination device with an approved safety barrier for current loop operation in a specific hazardous location. A 4-wire transmitter block diagram is shown in Figure 5 below. A notable item is that the 4-wire device itself uses a separate DC power supply for operation, just like a 3-wire transmitter, and supplies loop current that way.
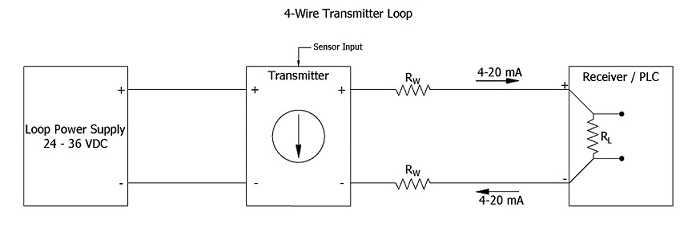
Figure 5 Typical current loop using a 4-wire transmitter
Review of 4-20 mA Data Transmission
The foregoing exposition has provided a brief description of the 4-20 mA current loop data transmission process. It is useful to conclude with a summary of the features and benefits of this process, as well as its limitations.
Advantages
- The 4-20 mA current loop is the dominant data transmission standard in many industries.
- It is recognized as the simplest analog data transmission method to connect and configure.
- It uses less wiring and connections than other methods, greatly reducing startup/setup costs.
- It is superior over long distances of field wiring, as current won't diminish, unlike voltage does.
- It is relatively insensitive to most electrical noise and related EMI (electromagnetic interference).
- It permits a local or a remote readout or monitoring device to be inserted in series with the loop.
- It is self-diagnostic of faults in the measuring system because 4 mA is equal to a 0% system output, so a loop current substantially lower than 4 mA becomes an immediate indicator of a system fault.
Limitations
- 4-20 mA current loops can only transmit one specific sensor or process signal per loop.
- Multiple loops are required for applications where there are many sensor or process outputs that must be transmitted. A lot of field wiring will be needed, which can lead to serious issues with ground loops if the independent current loops are not properly isolated from each other.
- Isolation requirements become exponentially more complicated with a larger number of loops.